Two highly precise measurement experiments disagree on how long neutrons live before they decay: does this discrepancy reflect measurement errors or point to a deeper mystery?
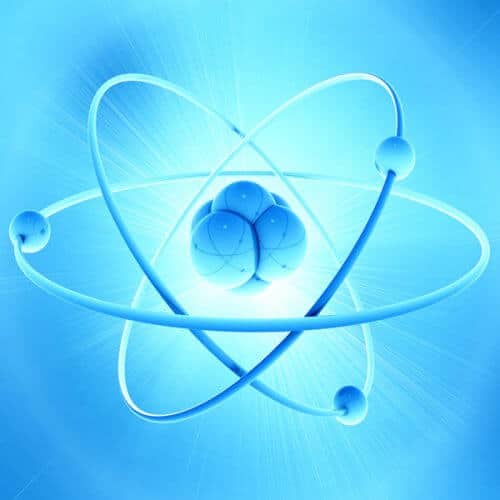
Geoffrey L. Green וPeter Glattenbort,
The article is published with the approval of Scientific American Israel and the Ort Israel network
in brief
- The best experiments in the world cannot agree on how long neutrons live before they decay into other particles.
- Two main types of experiments are carried out: bottle traps count the number of neutrons that survive after different periods of time, and beam experiments look for the particles into which these neutrons decay.
- Resolving the contradiction is essential to answer several fundamental questions about the universe.
Fortunately for life on earth, most of the material is not radioactive. We take this fact for granted, but in fact it is quite surprising, because the neutron, one of the two components of the atomic nucleus (in addition to the proton), is destined for radioactive decay. Inside an atomic nucleus, a typical neutron can survive for a very long time and may never decay, but when alone, it will turn into other particles within 15 minutes or so. The words "more or less" cover a disturbing gap in physicists' understanding of this particle. Despite our best efforts, we have not yet been able to accurately measure the lifetime of the neutron.
The "neutron lifetime puzzle" is not only a source of embarrassment for us experimenters, we must solve it if we want to understand the nature of the universe. The neutron decay process is one of the simplest examples of the "weak" nuclear force - one of the four fundamental forces in nature. To truly understand the weak force we must know how long neutrons live. Moreover, the length of time the neutron lasted determined how the lightest chemical elements were first formed after the Big Bang. Cosmologists would like to calculate the relative proportions of the elements and compare them to the astrophysical measurements: a match would confirm the theoretical picture, and a mismatch might indicate that an as yet undiscovered phenomenon affected the process. However, in order to make such a comparison we must know what the neutron's lifespan is.
Two experimental groups, one operating in France under Russian guidance and the other American, tried more than 10 years ago, each separately, to accurately measure life expectancy. one of us (Glattenburt) was a member of the first team, and the second (green) was a member of the second team. We and our colleagues were surprised and also a little disturbed to discover that there is a considerable gap between our results. Some theorists have suggested that the difference is due to exotic physics - that some neutrons in the experiment have become particles that have never been detected, a phenomenon that may affect the various experiments in diverse ways. But we suspected that the reason is much more routine: it is possible that one of the groups, or even both, simply made a mistake, or what is more likely - overestimated the accuracy of their experiment. The American team has now completed a long and painstaking project designed to test the main source of uncertainty in its experiment in hopes of resolving the discrepancy. But the hard work did not dispel the fog, but only confirmed our previous result. Similarly, other researchers later confirmed the findings of Glattenbort's team. This contradiction left us even more confused. But we are not saying despair - both groups and other researchers continue to search for answers.
schedule neutrons
Theoretically, measuring the lifetime of the neutron should be a simple and straightforward task. The physics of radioactive decay is well understood, and we have sophisticated methods for studying the process. We know, for example, that if a particle has the possibility to become a particle with a smaller mass or several particles whose sum is smaller, and in this process retain properties such as charge or angular momentum expressed in its spin, it will indeed do so. Free neutrons exhibit this instability. In a process called beta decay, a neutron decays into a proton, an electron, and an antineutrino (the neutrino's antimatter counterpart), three particles whose combined total mass is somewhat less than the neutron's mass, but the sum of their charges, spins, and other conserved quantities is the same as that of the parent neutron. These preserved quantities include the "mass-energy", that is, the daughter particles carry the mass difference in the form of kinetic energy, the energy of movement.
We cannot predict exactly when a particular neutron will decay because this process is a fundamentally random quantum phenomenon - we can only say how long neutrons live on average. Therefore, we must measure the average lifetime of the neutron by examining the decay of many neutrons.
The researchers used two experimental methods - one is called the "bottle" method, and the other is called the "beam" approach. Flask experiments confine neutrons inside a container and count how many remain after a given time. In the beam method, on the other hand, one does not look for the disappearance of neutrons but for the appearance of the particles into which they decay.
The bottle approach is particularly challenging because neutrons can easily pass through material and anyway also through the walls of most containers. Following a proposal first explicitly formulated by the Russian physicist Yuri Zeldovich, experimenters using the flask approach—such as Glattenburt and his colleagues in France—get around the problem by trapping very cold neutrons (that is, neutrons with very little kinetic energy) inside a smooth-walled container. Very [see illustration]. If the neutrons are slow enough and the bottle is smooth enough, they will be reflected from the walls and will therefore remain inside the bottle. To achieve such a result, the velocities of the neutrons have to be on the order of a few meters per second, as opposed to the speed of about 10 million meters per second, or so, at which they travel when they are emitted during nuclear fission, for example. These "ultra-cold" neutrons are so slow that you can outrun them. The most accurate bottle experiment to date was conducted at the Laue-Lanjevan Institute (ILL) in the city of Grenoble in France.
Unfortunately, no bottle is perfect. If neutrons occasionally leak out of the flask, we will attribute this loss to beta decay and get an incorrect lifetime. Therefore, we must make sure that we introduce a correction in our calculations so that we only take into account the particles that actually underwent beta decay.
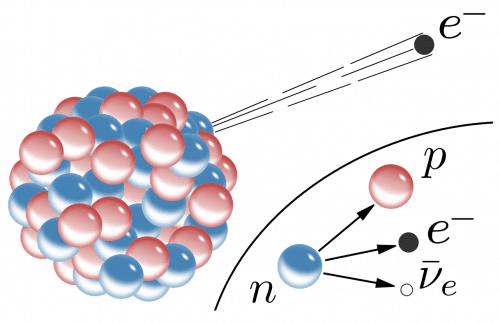
For this repair we used a sophisticated method. The number of neutrons that are lost through the walls of the flask depends on the rate at which the neutrons collide with the walls. If the neutrons are slower or the bottle is larger, the rate of collisions will decrease - and in any case, the rate of neutron loss as well. We performed a series of experiments during which we changed both the size of the bottle and the energy (and therefore, the speed) of the neutrons, and thus we could relate through extrapolation to a hypothetical bottle in which there are no collisions and in any case - there are no losses through the walls either. Of course, this extrapolation is not perfect, but we do our best to account for any errors involved in this calculation.
In the beam method - used by Green and others at the Center for Neutron Research at the American National Institute of Standards and Technology (NIST) - we sent a stream of cold neutrons through a magnetic field and a ring of high voltage electrodes that trap positively charged charges [see figure]. Neutrons are electrically neutral, so they pass straight through the trap unimpeded. However, if a neutron decays inside the trap, the resulting proton, charged with a positive charge, remains "stuck". At regular intervals we "open" the trap, remove the protons from there and count them. Basically, the capture of the protons and their detection are close to perfect, and we have to introduce only very slight corrections to compensate for the possibility that we have missed decays.
Where could we go wrong?
For a measurement to be useful, it must be accompanied by a reliable assessment of its degree of accuracy. Measuring a person's height with an uncertainty of one meter, for example, will give much less significant results than a measurement whose uncertainty is one millimeter. For this reason, when we make precision measurements we must always report the uncertainty of the experiment; An uncertainty of one second, for example, means that there is a high probability that our measurement result does not deviate from the true value by more than one second, up or down.
In general, any measurement has two sources of uncertainty. A statistical error arises because in an experiment it is possible to measure only a finite sample - in our case, a finite number of particle decays. The larger the sample, the more reliable the measurement and the lower the statistical error.
The other source of uncertainty - systematic error - is more difficult to assess because it is created due to deficiencies in the measurement process. These deficiencies can be something simple, such as measuring a person's height with a tape measure that is not properly calibrated, and they may be more subtle, such as a biased sample - in a telephone survey, for example, they may focus too much on calls to landlines rather than mobile phones, thus missing a sample A true representative of the population. The experimenters invest enormous efforts to reduce these systematic errors, but it is impossible to eliminate them completely. The best we can do is to conduct a detailed study of all imaginable sources of error and then estimate the effect each will have on the final result. We then add this systematic error to the statistical error to get the best estimate of the overall reliability of the measurement. In other words, we put a lot of effort into evaluating the "known unknown".
Of course, our biggest concern is that we lose sight of an "unknown unknown" - a systematic effect that we don't even know we don't know about - hidden within the experimental procedure. Despite all that we work hard to check all the possible uncertainties, the only way to overcome another error of this type with real confidence is to conduct a different and completely independent measurement using a completely different measurement method whose systematic effects are different. If two such measurements agree, within the uncertainty range calculated for each of them, then we can trust the results. But if they don't match, we have a problem.
To measure the lifetime of the neutron we have two such independent methods: the beam and the bottle. The latest result from the beam experiment at NIST for the neutron lifetime is 887.7 seconds. We determined that the statistical uncertainty in our estimate is 1.2 seconds and the systematic uncertainty is 1.9 seconds. A statistical addition of these two error ranges gives a total uncertainty of 2.2 seconds, and this means that we believe that the true value of the neutron lifetime is within 2.2 seconds of the measured value, with a probability of 68%.
In the flask experiment at the ILL, however, the measured neutron lifetime was 878.5 seconds with a statistical uncertainty of 0.7 seconds, a systematic uncertainty of 0.3 seconds, and a total uncertainty of 0.8 seconds.
These are the two most accurate experiments of their kind in the world to measure the lifetime of the neutron, and their results differ by approximately nine seconds. Such a time frame may not sound very large, but it is much larger than the uncertainties calculated for the two experiments - the probability of getting a difference of this size by chance is less than 1 in 10,000. Therefore we must seriously consider the possibility that the contradiction stems from an unknown unknown, that is, we have missed something important.
Exotic physics
This difference has an exciting possible explanation: that it actually reflects an exotic physical phenomenon that has yet to be discovered. One of the reasons to think that such a phenomenon may exist is that although the beam and bottle results do not match each other, other beam studies show a good agreement between themselves, and similarly also bottle studies.
Imagine, for example, that in addition to normal beta decay, the neutrons also decay through a so far unknown process, which does not create the protons that are being searched for in the beam experiments. The flask experiments, which count the total number of "lost" neutrons, will be able to count both the neutrons that disappear through beta decay and those that have gone through this other process. We can therefore conclude that the life span of the neutron is shorter than the life span which is only affected by "normal" beta decay. At the same time, the beam experiments will dutifully record only beta decays that produce protons and therefore yield a higher value for lifetime. So far, as we have seen, indeed the beam experiments measure a somewhat longer lifetime than that measured in the flask experiments.
There are some theorists who take this idea seriously. Zurab Barjiani from the University of L'Aquila in Italy and his colleagues proposed such a secondary process: a free neutron, they suggest, may sometimes become a "mirror neutron" that no longer reacts with normal matter and therefore will appear as if it has disappeared. Such mirror material could contribute to the total amount of dark matter in the universe. Although this idea is very exciting, so far it is nothing more than an unsubstantiated hypothesis. There is a need for a more unambiguous confirmation of the gap between the bottle method and the beam method for measuring the life span of the neutron so that most physicists will agree to accept such a revolutionary idea as a mirror material.
We believe that a much more plausible possibility is that one of the experiments (or maybe even both) did not correctly estimate a systematic effect, or overlooked such an effect. Such a possibility always exists when working with delicate and sensitive experimental setups.
Why is the neutron lifetime important?
The discovery of what we missed could of course give us, the experimenters, some peace of mind. But more importantly - if we can get to the bottom of this mystery and accurately measure the life span of the neutron, we may be able to deal with several fundamental and old questions about our universe.
First and foremost, an accurate estimate of the characteristic time for neutron decay will teach us how the weak force acts on other particles. The weak force is responsible for almost all radioactive decays and is the cause, for example, of the nuclear fusion that takes place inside the sun. Neutron beta decay is one of the simplest and purest examples of the weak force interaction. To calculate the details of other, more complicated nuclear processes involving the weak force, we must first gain a full understanding of how it works in neutron decay.
Determining the exact rate of neutron decay will also help us test the Big Bang theory that describes the early development of the cosmos. According to this theory, when the universe was about one second old, it consisted of a hot, compressed mixture of particles: protons, neutrons, electrons, and more. At that point, the temperature of the universe was about 10 billion degrees - so hot that the energy of these particles was too high for them to bind together to form nuclei or atoms. After about three minutes, the universe expanded and cooled to a temperature where protons and neutrons could stick together to form the simplest atomic nucleus, Deuterium (the heavy isotope of hydrogen). From there on, other simple nuclei could be formed - deuterium could capture a proton and form an isotope of helium, two deuterium nuclei could fuse to form a heavier helium, and small amounts of smaller nuclei were formed, up to the element lithium (all the heavier elements were formed, according to the accepted theory , within stars, millions of years later).
This process is called Big Bang nucleosynthesis. If, when the universe lost heat, neutrons would have decayed at a rate much faster than the rate of cooling of the universe, there would have been no neutrons left at the stage when the universe reached a temperature suitable for the creation of nuclei - only protons would have remained, and we would have received a cosmos made almost entirely of hydrogen. On the other hand, if the life span of the neutron was much longer than the time required for cooling that enables big bang nucleosynthesis, the result would be a universe with too much helium, which in turn would affect the creation of the heavier elements involved in the development of stars and ultimately - the development of life. It therefore turns out that the balance between the cooling rate of the universe and the life span of the neutron is a decisive factor in the creation of the elements that make up our planet and everything on it.
Astronomical data allows us to measure the cosmic ratio between helium and hydrogen, as well as the amounts of deuterium and other light elements present throughout the universe. We would like to see if these measurements are consistent with the numbers predicted by the big bang theory. However, the theoretical prediction depends on the exact value of the neutron's lifetime. Without a reliable value for this lifespan, our ability to make a comparison is limited. Once we know the neutron lifetime more precisely, we can compare the observed ratio we got from astrophysical experiments with the value predicted by theory. If they match, we can feel more confident in our standard Big Bang theory scenario of how the universe evolved. Of course, if they don't fit, we may have to change this model. For example, certain contradictions may dictate the existence of new exotic particles in the universe, such as another type of neutrino, which could interfere with the nucleosynthesis process.
One of the ways to reconcile the difference between the beam experiment and the bottle experiment is to conduct more experiments using methods with similar precision, which are not subject to the same systematic errors that may confuse us. Alongside the teams that continue to conduct Bottle and Beam projects, scientists in other groups around the world are working on alternative methods to measure the lifetime of the neutron. One group at Japan's Joint Proton Accelerator Facility (J-PARC) in Tokai is developing a new beam experiment that will detect electrons rather than protons produced in neutron decay. In another exciting development, groups at the ILL, the St. Petersburg Institute of Nuclear Physics in Russia, the Los Alamos National Laboratory, the Technical University of Munich and the Johann Gutenberg University of Mainz in Germany plan to use neutron bottles that will confine ultracold neutrons using magnetic fields instead of material walls. This is possible because the neutron, although electrically neutral, behaves as if it were a small magnet. The number of neutrons that will accidentally be lost through the sides of such bottles should be completely different from their number in previous experiments and therefore the systematic uncertainties that will accompany the method will also be completely different. It is our fervent hope that this combination of the continued flask and beam experiments with this new generation of measurements will finally solve the neutron lifetime puzzle.
3 תגובות
A. Ben Ner
We know the reason for non-disintegration of neutrons inside atomic nuclei. The reason is energy considerations. When a neutron decays, a proton is created. Because there are other protons in the nucleus - the new proton must have a higher energy (Pauli's exclusion principle).
From a scientific point of view - this discrepancy is a great thing. There is a reason for this discrepancy, and by studying the reason (or the complication), we will learn new things. There are many discoveries in science that are the result of inconsistencies in the results of experiments.
Perhaps the shorter half-life in the bottle experiment can be explained by the existence of pairs between the neutrons in the experiment (see the article in Science "Discovery sheds new light on what happens inside the atomic nucleus and in neutron stars" dated August 15, 2018). The decay of one neutron from a pair will surely increase the momentum of the partner. This does not affect the results in the beam method, because protons are counted there, but in the bottle method the partner can gain enough momentum to exit through the wall.
I have an idea regarding the problem at hand.
The idea is as follows: in the beam experiment, an electric field and a magnetic field are activated.
These fields affect and reduce the neutron decay rate.
This hypothesis is supported by the well-known fact that the decay rate of neutrons in the nuclei of atoms is almost zero.
We must therefore ask, what is the factor in the atomic nucleus that so radically changes the neutron decay rate?
The hypothesis is that the cause is the electric field and possibly also the magnetic field that the protons impose on the neutrons in the atomic nucleus.
If this hypothesis is indeed true, then there is a dependence between the electromagnetic force and the weak nuclear force.
This connection is not a theoretical innovation and it already exists, as a hypothesis, in the theory of the high energies, which describes the 4 fundamental forces in nature as splits of one ancient fundamental force, which existed in the universe in the most initial stages after the big bang, under conditions of fairly high temperature, pressure and density.
(Note: it is so possible that this one ancient force is not only ancient but also exists in the present within the horizon of black holes).
The caveat, however, is that the split between the weak nuclear force and the electromagnetic force is not a complete split and it is possible that these two forces are different manifestations of one fundamental force, two sides of the same coin.